Nick Eyre, Sarah J. Darby, Philipp Grünewald, Eoghan McKenna and Rebecca Ford
Summary
A 1.5°C global average target implies that we should no longer focus on merely incremental emissions reductions from the electricity system, but rather on fundamentally re-envisaging a system that, sooner rather than later, becomes carbon free. Many low-carbon technologies are surpassing mainstream predictions for both uptake and cost reduction. Their deployment is beginning to be disruptive within established systems. ‘Smart technologies’ are being developed to address emerging challenges of system integration, but their rates of future deployment remain uncertain. We argue that transition towards a system that can fully displace carbon generation sources will require expanding the focus of our efforts beyond technical solutions. Recognizing that change has social and technical dimensions, and that these interact strongly, we set out a socio-technical review that covers electricity infrastructure, citizens, business models and governance. It describes some of the socio-technical challenges that need to be addressed for the successful transition of the existing electricity systems. We conclude that a socio-technical understanding of electricity system transitions offers new and better insights into the potential and challenges for rapid decarbonization.
Red block version
1. Introduction
(a) Rapid decarbonization
Delivering any acceptable target for long-term warming requires rapid transformation of global energy systems that are responsible for most greenhouse gas emissions. Decarbonization of the electricity sector is crucial and an early action is needed. Of course, electricity decarbonization is not the entire problem for energy transition. However, there is general agreement that mitigation in other sectors will be more difficult and also that their decarbonization may require a degree of electrification. A whole-systems approach shows that early decarbonization of electricity is essential for both reasons. This paper therefore focuses on that challenge for electricity systems.
A critical effect of shifting attention from a 2°C target to a 1.5°C target will be the need for faster energy system transformation, and therefore even higher priority for rapid electricity system transformation. However, our analysis is applicable to the rapid transformation required to prevent dangerous anthropogenic climate change in general, rather than any specific temperature target.
(b) Integration of renewable energy
Variable (intermittent) renewables, in particular wind and solar, are now almost certain to be the major contributors to low-carbon electricity supply. They are abundant resources and the lowest cost low-carbon options almost everywhere. Nuclear power and fossil fuel generation with carbon capture and storage (CCS) have featured prominently in earlier discussion about electricity decarbonization, including the IPCC AR5 report. However, it is increasingly clear that they cannot compete on cost with renewables, that they have other non-carbon environmental risks, and their development and build times are long. They are less suitable supply options in scenarios requiring very rapid decarbonization.
A number of global model outputs include high levels of electricity generation from a negative emissions technology, bio-energy with carbon capture and storage (BECCS), by the second half of this century. However, these modelling processes have not fully considered the environmental, social and governance issues, which may be very problematic. More importantly, for the purposes of our analysis, the technology is virtually unproven and cannot be feasibly scaled to make a significant global contribution on the timescales required for electricity system decarbonization. Whether BECCS can play a major role in the long term or not, the first priority is rapid decarbonization.
At the rates of decarbonization implied by a 1.5°C (or even a 2°C) target, the scale of deployment of renewables is therefore a key challenge. Reducing total demand (below the counterfactual) will therefore be critical in constraining this scale and therefore increasing the speed of decarbonization. There is a general agreement between integrated assessment models on this, and also with other bottom-up assessments.
The key problem for a rapid renewables transition is to integrate these variable resources into existing power systems. These challenges are already appearing in countries with high renewables penetrations; integration is increasingly the key constraint on speed of deployment.
Variable renewable resources are also more distributed than conventional fossil-fuelled power plants and many are connected to power systems at lower voltages, so that grid and system operation need to adapt.
(c) Electricity decarbonization as a socio-technical issue/challenge
This paper considers the challenges of a major transition to a low-carbon electricity system powered by renewables. Although most of the literature on climate mitigation focuses on energy supply, there is increasing recognition that energy demand will also play a role. Indeed, energy efficiency improvement has historically played a larger role in mitigation than supply decarbonization and sectoral analyses indicate that there is still a very large potential.
Energy demand is highly distributed and the outcome of interactions between people and technology. The ‘demand side’, and human actors more generally, cannot be neglected in thinking about low-carbon transitions. Major transitions involve co-evolution of technology, society and institutions, both generally and specifically for electricity. Technology invention, development, adoption and adaptation involve individual, social and organizational learning. A technology, and still more a system, is never fully ‘achieved’ or ‘delivered’: it is continuously adapted in response to prevailing conditions.
The role of an electricity system is to provide services for human activity and well-being. In a renewables-based electricity system, important human dimensions will include service expectations, knowledge and skills, technology acceptance (e.g. of extensive renewable generation, transmission lines and smart metres) and more active citizen engagement, for example in the deployment of decentralized generation (DG), distributed storage (DS) and demand response (DR). In this sense, technical change is ‘an irredeemably social process’ and it is unhelpful to draw boundaries between the social and technical, hence the socio-technical approach that we adopt in this paper.
It is also important to understand that decarbonization is not the only energy-related social and political priority, nor even the main one in most jurisdictions. Access to electricity is a key sustainable development goal, and the key energy policy goal in many developing countries. Where access can use new, cleaner technologies thereby ‘leapfrogging’ the technologies of more industrialized countries, climate mitigation opportunities may be advanced. Where there is already universal access to electricity, security of supply is usually the critical political concern, with affordability also a key political goal, for both households and businesses. Universal access to electricity and affordable security of supply are therefore critical constraints on, and drivers of, any proposed transition.
2. Four categories of change
Transitions tend to involve three categories of human actor—citizens, businesses and governance actors. In addition to these human actors, energy transitions are subject to path-dependency created by long-lived and capital-intensive physical infrastructure. Most notably, developed countries have electricity transmission and distribution infrastructure that was designed and built for large centralized generators to serve distributed and ‘passive’ loads. This socio-technical ‘lock-in’ has implications for future transitions. Distributed generation and control challenge these established systems and their operations. Given the socio-technical nature of the decarbonization challenge, we treat the physical infrastructure on equal terms with human actors in the three roles identified above, analysing transition requirements around these four interacting categories (Figure 1):
- (a) physical infrastructure (grids/distribution networks and systems),
- (b) consumers/citizens,
- (c) energy business models and
- (d) institutions and governance.
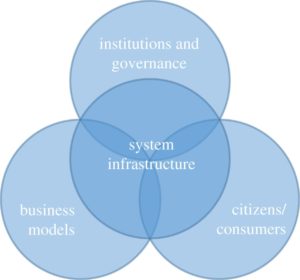
The following sections describe the challenges of transitioning to a zero-carbon electricity system for these categories.
(a) Infrastructure
Wind and solar power can provide electricity that is sustainable, increasingly affordable, and can be deployed rapidly at scale. These resources therefore provide the best prospects for a rapid low-carbon electricity transition. Energy security, however, requires balancing the supply and demand of electricity. Wind and solar power depend on sources of energy that are variable and cannot be controlled. Although significant amounts of variable generation can be adopted without problems, it is self-evident that moving towards very high penetrations of variable resources raises balancing challenges. System balancing is one of the most fundamental challenges of transitioning to zero-carbon electricity systems.
The variability of net demand (electricity demand minus non-controllable renewable electricity supply) is a critical issue. Net demand needs to be balanced on multiple timescales from seconds to seasons. Technically the key options for integrating variable renewables are known: flexible generation, storage, network enhancement (incl. interconnection) and flexible demand.
Flexible generation is the best established and currently the most widely used. However, at very high levels of variable generation, it implies the construction of very large amounts of flexible plant, much of it with very low-load factors, which makes them risky assets in which to invest. Furthermore, renewable electricity (other than biomass) has very low short-run marginal costs. This exacerbates the ‘missing money problem’ in classical wholesale markets, where generator revenues cannot cover investment in new capacity to ensure reliable supply. This has already impacted markets in a number of countries. The problem can be addressed in a number of ways, including by capacity markets. However, it also increases the need for flexibility. The ease with which fossil fuels can be stored and the relatively low cost of capacity have resulted in flexibility being readily available from thermal generators. The closure of such plant reduces system inertia (rotating mass of generators) and load-following ability at a time where variability of net demand is likely to increase. The best and cheapest approach to close this gap will involve thinking wider than supply.
Energy storage is an alternative. Batteries, for example, are already being deployed at scale to provide ancillary services to grids. The energy to power ratio of batteries makes them well suited to balancing over relatively short timescales e.g. seconds, hours and, possibly, days. While the cost of batteries is still high, it is falling rapidly and battery deployment could see considerable growth. Batteries are, however, unsuitable for dealing with the long-term balancing (multiple-day, seasonal) also required in high renewables scenarios. Storage options for long-term balancing require high energy-to-power ratios and low energy costs. Chemical and thermal options are more plausible than batteries. Hydrogen storage has suitable characteristics but requires considerable research and development to reduce costs. In low-carbon electricity systems, energy storage can provide an effective solution to short-term balancing, but the technologies for cost-effective long-term balancing are not proved.
Energy storage can balance net demand by shifting energy in time. Networks do the same by shifting energy in space. This is of benefit where the net demand time profiles of adjacent systems differ, so that their inter-connection aggregates their net demand and reduces its variability. At a large scale, interconnection is costly but has an important role in balancing short-term variability of net demand. Distance of interconnection is roughly analogous to duration of storage. Balancing using interconnection alone might imply trans-global interconnection: east-west connection for diurnal balancing and north-south for seasonal. The costs and geo-politics of such networks make them unlikely.
In addition, there is a need for ‘smarter’ low-voltage networks. In most jurisdictions, these are still predominantly passive (non-smart). Increased exposure to reverse flows from distributed generators and more variable large loads (such as electric vehicles) both increase the need for more active demand management at the low-voltage network level, which requires changes in their control systems and the role of the network operators themselves.
The final option, flexible demand, turns the traditional ‘predict and provide’ paradigm of power systems on its head, with demand responding instead to changes in supply. Evidence from existing dynamic electricity pricing schemes demonstrates that DR can play a useful, if currently limited, role in managing short-term inter- and intra-day variability. There is considerable research and development into how flexible demand can play a larger role in balancing, however, mainly focussed on electrifying domestic heat and transport and using these as distributed thermal and electro-chemical storage.
While these options can help reduce variability of net demand over short timescales, the electrification of heat, in particular, may increase the long-term seasonal balancing challenge, further increasing requirements for long-term seasonal flexibility. Until the costs of long-term storage technologies drop, it remains critical to encourage demand reduction, through energy efficiency and lifestyle changes. This requirement for both flexibility and reduction implies considerable changes to the status quo.
It is important to note that all the newly built infrastructure on the demand side that a highly distributed smart grid implies has considerable potential to pre-shape behaviour and lifestyles in ways that may or may not support demand reduction or flexibility efforts. For example, households adopting solar panels in different countries can end up with quite different behavioural cues depending on the particular metering configurations and subsidies in place in their country. Early adopters of PV in Germany were incentivized to shift demand outside of daylight hours to export as much of their generation as possible, while the opposite has always been true in the UK. Meanwhile, solar adopters in the USA under net metering arrangements are not incentivized to time-shift demand at all. Despite the large number of installations worldwide, the overall impact of solar PV on household energy consumption behaviour and how this varies socio-demographically is not well understood, but is illustrative of the (sometimes unintended) behavioural consequences of low-carbon infrastructural changes on the demand side.
(b) Consumers and citizens
The traditional (usually implicit) conception of an electricity consumer is as a passive recipient and purchaser of a commodity (the kWh), and someone whose choices and actions are limited to (a) switching electrical equipment on and off, and (b) a choice of tariff and, perhaps, a choice of supplier. As innovations in demand or supply are expected to be technical or regulatory in nature, the consumer’s role is a largely reactive one: to invest in new technology and adapt to new infrastructure or market arrangements.
This account of consumers is challenged when we consider the extent to which electricity systems rely on networks of distributed actors, their role in shaping demand. This is becoming more significant as electricity systems increasingly involve distributed resources, control, network infrastructure, participation and governance. The socio-technical energy research literature points to the dangers of assuming that consumers are passive participants in energy systems or (worse) that they are error-prone obstacles in the path of technical progress. This approach tends to assume that human understanding and activity can be ‘edited out’ of energy management, for example through highly automated smart homes, although the evidence to date is that this is often ineffective and can be counterproductive. The growing body of research into energy systems as socio-technical entities offers constructive and flexible ways of recognizing and analysing relationships between people, technology and activities.
Electricity demand is shaped by the demand for the services it provides. In a number of countries, notably in the EU, electricity consumption has fallen over recent years. Technological improvements, such as more efficient appliances and lighting, have undoubtedly been important, but routine activities and expectations for energy services also have to be taken into account when attributing changes in demand. Changes are not simply a matter of individual choice either. For example, in Japan, more relaxed dress codes for office workers in summer have had a considerable impact on demand, particularly at peak times and there have been substantial, durable demand reductions in the wake of the Fukushima disaster. Social norms and citizen-like responses to supply limitations both played a major part in bringing about these changes. The analysis of energy system evolution also shows the significance of networks of actors who between them bring about change or maintain the status quo: some striking recent analyses highlight the ways in which ‘middle actors’ may have the agency and capacity to bring about change because of their position in energy systems and the knowledge and connections they can bring to bear on a situation.
These considerations demonstrate the centrality of human actors in electricity systems and also some ways in which humans are more than passive consumers, even in relatively traditional systems. As they become more highly distributed, the operational challenges are likely to increase somewhat: systems will need to cope with changes in demand, supply mix, physical elements, rules and personnel over timescales ranging from milliseconds to decades. This will need reformed governance (discussed below) and that implies a need to recognize energy users as contributors and citizens, not simply consumers.
The nature of citizenship/involvement ranges from demand management by individuals to highly sophisticated forms of prosumption, community energy and involvement in planning issues. The dramatic rise of distributed generation in recent years, especially solar photovoltaics, has resulted in a whole new category of actors, prosumers, who are both consumers and producers. There is some evidence that prosumption increases with a sense of energy citizenship. To date, there is less experience with distributed electricity storage, although mass market products are now being sold. DR is a growing phenomenon, first practised only with major industrial electricity users but now involving small organizations and individual consumers. The spread of smart metering makes DR viable at scale, whether this takes place in response to dynamic pricing or a knowledge of system conditions, or through direct external control of some loads. The importance of trust between customer and supplier, customer education and feedback and user-friendly tariffs and technology is highlighted by a number of authors: effective DR emerges from constructive relations between consumers-as-citizens, suppliers and system operators, technologies, knowledge and skills.
Given the need for major increases in the deployment of energy efficiency measures, DR, distributed generation and DS, urgent change is still needed in the high-consuming economies. While the trend towards more distributed assets, activity and control in electricity systems is generally welcomed as part of a low-carbon transition, it raises important equity issues. In many countries, distributed generation seems likely to be a key enabler of cost-effective access to electricity. But, there are also risks associated with DG in countries with established grids, notably of rising network charges leading to ‘grid defection’ by customers able to invest in their own generating capacity, and prohibitive costs of supply for others who are trapped between the high cost of maintaining a residual grid and the cost of ‘going it alone’. There is also the risk in hot climates that the growth in air-conditioned buildings will produce dysfunctional systems that are unable to cope with peak demands and with pronounced inequalities between those who can afford high standards of service and those who cannot and who may, moreover, be suffering from ‘heat island’ effects exacerbated by air conditioning. A key question is whether new business models evolve to capture benefits primarily for individuals or for wider society. The former could lead to serious equity challenges and overall higher system costs (increasing the risk of ‘grid defection’), while the latter raises questions about cost/benefit attribution. The concept of energy citizenship is relevant in this debate.
A smart grid—that is, an electricity grid combined with an ICT network—allows system balancing (technical operation), time-dependent price signals (commercial operation) and real-time information to assist user engagement (social operation). Smart meters with the ability to measure and communicate consumption and generation in near-real-time are increasingly being deployed in many countries for various reasons, including enabling DR, DG and DS. There is some evidence of benefits in terms of better customer feedback, reduced demand and DR in countries with metering programmes designed to achieve these. However, there are also substantial challenges, including data security/privacy and cyber-security.
The scale of the potential for DR that could be enabled by smart grids is large and there is potential for such developments to enable capacity and flexibility goals to be met by distributed resources. However, significant uncertainty remains about the extent to which this potential can be realized, given the many geographical, social, physical and regulatory variables involved. Smart data and ICT solutions by themselves do not deliver increased flexibility, but merely enable access to flexibility that may or may not be forthcoming from energy service users. A smart washing machine is still subject to social schedules, conventions and preferences that dictate its operation, sometimes within highly constrained boundaries. The desired flexibility might therefore be less constrained by technology than by social norms and conventions, such as working hours and dress codes. Issues like this cannot be addressed when energy users are thought of purely as consumers; as citizens and actors, though, they take their place as agents in transition.
(c) Business models
The business model of the traditional utility (or vertically integrated electricity retailer) is increasingly problematic. The growth of the ‘missing money’ problem has had observable business impacts, with declining profitability for incumbent companies. As reliance on wholesale market prices becomes increasingly problematic, there is a need, and the potential, to look at the many new value streams in a decarbonized system. Some of these are in electricity markets, e.g. capacity, flexibility and the potential to address grid congestion, where demand side investments can contribute, for example, through DR, DG and DS. Some others investments are, by definition, on the demand side, for example, in end-use efficiency, heating and transport systems. All of these need to be financed, manufactured, installed, operated. So, there is a wide diversity of potential new business models in decarbonizing the electricity system, and the required size of some is large.
Electric utilities and vertically integrated generation/retailers are the traditional aggregators of energy demand. Future markets seem likely to require similar aggregation of capacity and flexibility resources from decentralized producers, i.e. of DG, DR and DS, which offer potential value streams to energy users, grid companies and system operators. In principle, the incumbent companies could develop their business models to incorporate new value streams, even without being regulated to do so, as these new services do not necessarily reduce demand for the core business kWh commodity. In practice, new entrants such as Sonnen, Tesla, Stem, Sunverge and Green Charge Networks, have largely taken the lead, due to their more flexible business practices. However, incumbents retain the advantages of a large customer base and expertise in wholesale markets, so it would be unwise to think that they will easily give way to new entrants. Many leading European energy companies, notably E.On, Innogy and DONG, have changed, or are changing, their fundamental business models towards a stronger focus on renewable generation and grid development. They are also including value-added services to their existing customers, particularly around DR, which requires relatively limited investment and is amenable to central control, and thus is relatively easy to add onto existing energy retail models.
However, the possibility of more radical changes in energy retail markets cannot be excluded, with conventional aggregation of small user demand supplanted by virtual platforms and ‘peer-to-peer’ trading, in the way that is increasingly familiar for services as diverse as taxis, second-hand goods and accommodation. In principle, these ICT-enabled approaches could ultimately replace the wholesale market entirely.
The value of electricity storage will rise in high renewables systems. Traditionally, pumped hydropower has been used for meeting peaks, but its potential is limited in the context of future balancing challenges. Gas has long been identified as a bridging fuel in decarbonization, initially because of its relatively low-carbon content and more recently as a complement to renewables in power generation. Incumbent utilities, as well as new entrants, have moved into gas-fired generation in many countries since the 1990s. However, the long-term role of unabated gas is limited in very low-carbon scenarios. Biomass and hydropower can play significant roles in some jurisdictions, but have constrained potential globally, and with the larger challenges in predominantly wind/solar systems, storage has several potential values, including wholesale market arbitrage, system services and grid support.
Early applications of storage have been in high-power applications, notably for fast frequency response, with incumbents as the key actors due to their ability to manage large projects and operate in complex markets. While the economics of wholesale market arbitrage are also improving rapidly, the relative attractiveness of centralized storage and DS remains unclear, and will probably be dependent on the details of DG support mechanisms and distribution charging tariff structures. Based on the experience of PV investment, it seems highly likely that new entrants with new business models will be better placed than incumbents in distributed applications. The rapid deployment of batteries in EV opens up the prospect of new business models at the interface of transport and electricity systems. Existing EV lithium-ion battery technology may not prove ideal in grid applications; however, new technologies are possible and the use of ‘post-vehicle life’ batteries offers an alternative business model.
For the reasons set out above, it seems unlikely that electro-chemical storage can address all the balancing issues associated with wind intermittency and seasonal demand. Chemical and thermal storage options are more plausible, but the short-term business models associated with them are in technology development and demonstration rather than in the mass market.
Collectively, distributed solutions imply billions of investments and the expected scale of demand side investment significantly exceeds that of new energy supply. The bulk of this will be in the relatively unglamorous areas of improving efficiency in buildings, transport and industry. Although there is some successful experience of energy companies diversifying into energy services offerings in the commercial and industrial sectors, it remains a relatively small market. Incumbent electricity system companies may be badly placed to undertake downstream financing, manufacturing, installation and operation, but that implies the need for new actors with new business models. Most investment has traditionally come from business and household users themselves. How this can be scaled to the required levels remains a challenge.
(d) Institutions and governance
There is a significant concern about the ability of existing governance structures to enable rapid change, with respect to climate mitigation in general and in particular electricity systems in existing market models, including about the ability of existing arrangements to deliver the expected levels of investment, innovation and engagement. So, it is clear that change is needed.
There is no panacea as the institutional frameworks differ greatly across countries. Resource geographies differ substantially; approaches designed for monopoly utilities (e.g. in the USA) cannot be necessarily applied in competitive retail market structures (e.g. in Europe); most importantly, the challenges of electricity access, affordability and organizational capacity faced by developing countries differ hugely from the issues that are grounded in well-established power systems. Further research is required to address the governance implications of all these factors. However, it is clear that there are some important common factors across different systems.
Already the penetration of technologies with very low marginal cost is leading to concerns about market incentives for adequate generation investment. Current responses by policy-makers, for example incentives for new capacity, do not generally address the need for much greater flexibility to accommodate variable generation. Most existing flexible generation is not low carbon, pointing to the need for more emphasis on flexible demand, grid investment and storage. The prospect of a rapid shift to a high penetration of variable renewables adds to these concerns. The major investments required in flexibility lie outside the area of expertise of industry incumbents. Systems are increasingly complex, multi-scalar and variable in real time. Some potential non-traditional business models, e.g. peer-to-peer trading, seem to imply radical changes in market structures, and therefore of governance.
The political importance of the electricity sector is such that, even in liberalized energy markets, governments cannot relinquish influence over key strategic decisions. Where market rules and codes are set by incumbents, rapid change is problematic. Governance and regulatory systems will need to encourage innovation at rates historically unknown in the sector. Neither traditional integrated utilities nor oligopolistic liberalized markets in electricity as a commodity are equipped to do this. Both renewable generation and its flexible complements are more distributed than existing centralized generation. Many new assets will be on the ‘customer side of the meter’, implying the need to engage billions of actors in a manner that assists system security and transition. This constitutes a major governance change.
Theories of energy regulation since the ‘liberalization’ agenda of the 1990s have focused on incentivizing cost reduction in networks and creating competition in generation and (in some jurisdictions) retail of electricity as a commodity. In the context of requiring rapid change, decentralization and greater flexibility, this is no longer fit for purpose. Public goods are now critical goals and private costs. Governance will need to enable low-carbon innovation. In practice, this implies reform will need to enable change in the other three areas set out above.
In the grid infrastructure, the operators need to move from being passive ‘wires companies’ to become active operators of their local networks, essentially downscaling the idea of system operation to the distribution level. This will require use of ‘smart grid’ technology to address the increasing imbalances (spatial and temporal) across their networks as new technologies and practices are adopted. This implies major changes in regulatory objectives to promote innovation, whether the network is unbundled or part of a larger utility. And it will involve enabling network companies taking a more active role (either by investment or incentivization) in DG, DR and DS approaches.
For consumers and citizens, the over-arching need is to create a framework that reverses the historical trend towards the passivity and disengagement associated with commodity supply. Trust in energy institutions needs to be re-established. Market rules need to promote consumer engagement in the new flexible technologies. Governance structures need to allow for effective representation of the interests of communities, citizens and new market actors.
Redesigning regulatory systems for new business models is not straightforward, but is essential. Consumer protection will remain important, but it needs to be proportionate. Routes for new business model approval should be transparent. And regulation should ensure that non-profit organizations are treated appropriately rather than necessarily under the rules designed for profit-making enterprises.
Over-arching all of these, the assumption that electricity systems are inevitably large scale, and therefore appropriately governed at national or state level, needs to be revisited.
3. Discussion
We have introduced four categories of change in turn. The interdependence and interactions between these categories have become evident throughout. In this section, we illustrate the need for whole-system consideration of these categories by way of an example. DR, efficiency and distributed generation have been referred to as cases with particular need for such a system-wide approach. Here, we will illustrate the multifaceted challenges and breadth of opportunities emerging from this perspective with the case of storage. Electricity storage, whether distributed or centralized, touches on all four of our categories.
Governance of storage is still developing. Established structures have evolved for systems which operate efficiently without explicit provision of storage. Issues of generation, networks and demand tend to be addressed separately, whereas storage cuts across these domains. In some cases, this separation is such that regulation actively inhibits storage. Where storage is classified as a generator, network operators, for instance, can be actively prohibited from owning or operating storage. Opportunities exist to re-define the role of storage and thus encourage its uptake as an integral system component.
Electricity markets have been developed predominantly to ensure the provision of energy, rather than storage per se. Business models therefore face a challenge of extracting value for storage from a range of markets (energy, capacity, response services) and non-markets (avoided infrastructure costs, long-term system integration, operation and decarbonization costs). Efforts to allow storage and DR to trade on ‘a level playing field’ alongside other generating assets fail to recognize the integrating role such new solutions contribute to the wider system beyond mere ‘generation’. Fostering business models with this wider scope could unlock significant change in the provision, distribution and re-shaping of electricity demand through various forms of storage.
The relationship of citizens with storage is multifaceted. Citizens could become owners and even operators of privately owned storage, if governance structures and new business models developed to make this an attractive option.
The allocation of the collective cost/value of storage may, however, also pose challenges for equity, as mentioned above. Without appropriate incentives, self-sufficient consumers with personal storage could withdraw support for collective system infrastructures, leading to a less efficient system where balancing takes place at an inappropriately small scale.
Conversely, centralized large-scale collective storage solutions and associated infrastructures, such as for hydrogen as a storage vector, pose similar challenges of equity. How should the cost be borne and distributed? To what extent should users of electricity with a load profile that heavily draws on storage be made to bear the cost, or those who mitigate the need for storage through more flexible demand patterns be rewarded? These questions lie at the interfaces of governance and business models, affecting citizens and infrastructure alike.
Far from a purely technical challenge, storage emerges as one of many solutions for a rapidly decarbonizing system, which benefit from the inclusion of human actors, be they in governance, business or civil society. The value of storage can be better understood in relation to the wider system and the benefit it brings to society. In this perspective, governance structures play an important role in enabling new business models, which, in turn, could unlock new levels of citizen engagement or infrastructure development.
Although there are huge socio-technical challenges, there are signs that we can be cautiously optimistic about addressing them. Generation and some infrastructure costs are falling, new businesses emerging, public engagement is strengthening, and in some places there are some effective policy drivers.
Electricity will not be alone in facing these issues in a low-carbon transition. There are analogies in systems that are more problematic for decarbonization, for example transport systems and food systems. Demand, lifestyle and social engagement in system change are common features.
This paper has argued the inseparability of technical and social aspects of energy systems. Significant progress has been made with ‘stand alone’ technologies in terms of cost and deployment. Looking forward, the role of civil society, businesses and governance in shaping the transition towards decarbonized electricity systems will become increasingly important.
4. Conclusion
We have discussed four interacting components for a transition towards a low-carbon electricity system: infrastructure, citizens, business models, institutions and governance. The interactions between these components highlight the socio-technical nature of the system transition ahead. We have shown that this perspective not only highlights the new opportunities for rapid decarbonization, but also raises issues that have not received sufficient attention in a predominantly technology-focused discourse.
The usual preoccupations of low-carbon studies are the major uncertainties in technology performance and cost, but there are other equally significant determinants of system change.
Electricity system scale and flexibility are likely to be critical and both involve decisions that are highly social in nature, relying as they do on complex commercial and operational arrangements and on the type and timing of activities that will be promoted or discouraged. Storage of various kinds is likely to be very important, and the required scale, location, durations and other performance requirements are strongly dependent on wider system flexibility, including the availability of DR. The technical potential of DR is clearly large; however, the adoption and realization of this potential depends on social factors, some of which currently do not form part of energy policy. The practical (i.e. socio-technical) potential has been the subject of conjecture but, as yet, remains uncertain.
Feasibility of a rapid low-carbon transition will depend on development of the human part of the energy system as least as much as its technical components, notably on smart grid deployment, electricity business model change, engagement of electricity users and governance arrangements. The relative roles of distributed and centralized actors are not yet clear, but significant decentralization seems inevitable. The implication is that although there are some geo-political constraints on energy transition, there is much scope for national and sub-national action, not least where electricity systems are concerned. This seems consistent with Paris Agreement approach of using Nationally Determined Contributions.
Funding
We further thank EPSRC for funding (EP/M024652/1).
Acknowledgement
The authors gratefully acknowledge funding from the Oxford Martin School through the Oxford Martin Programme on Integrating Renewable Energy. The Oxford Martin School is a world-leading centre of pioneering research that addresses global challenges. Visit: www.oxfordmartin.ox.ac.ukOpens in a new tab for more information.
Publication details
Eyre, N., Darby, S.J., Grünewald, P., McKenna, E. and Ford, R. (2018) Reaching a 1.5°C target: socio-technical challenges for a rapid transition to low-carbon electricity systems. Philosophical Transactions of the Royal Society A, 376. doi: 10.1098/rsta.2016.0462Opens in a new tab
Banner photo credit: Alireza Attari on Unsplash